Astronomical Scales, from City-Stars to Stagging "-illions"
by Adam Hadhazy
A fascinating observation of a tiny star hurling out a beam four trillion times its size offers an intriguing glimpse into the range of distances astrophysicists deal with
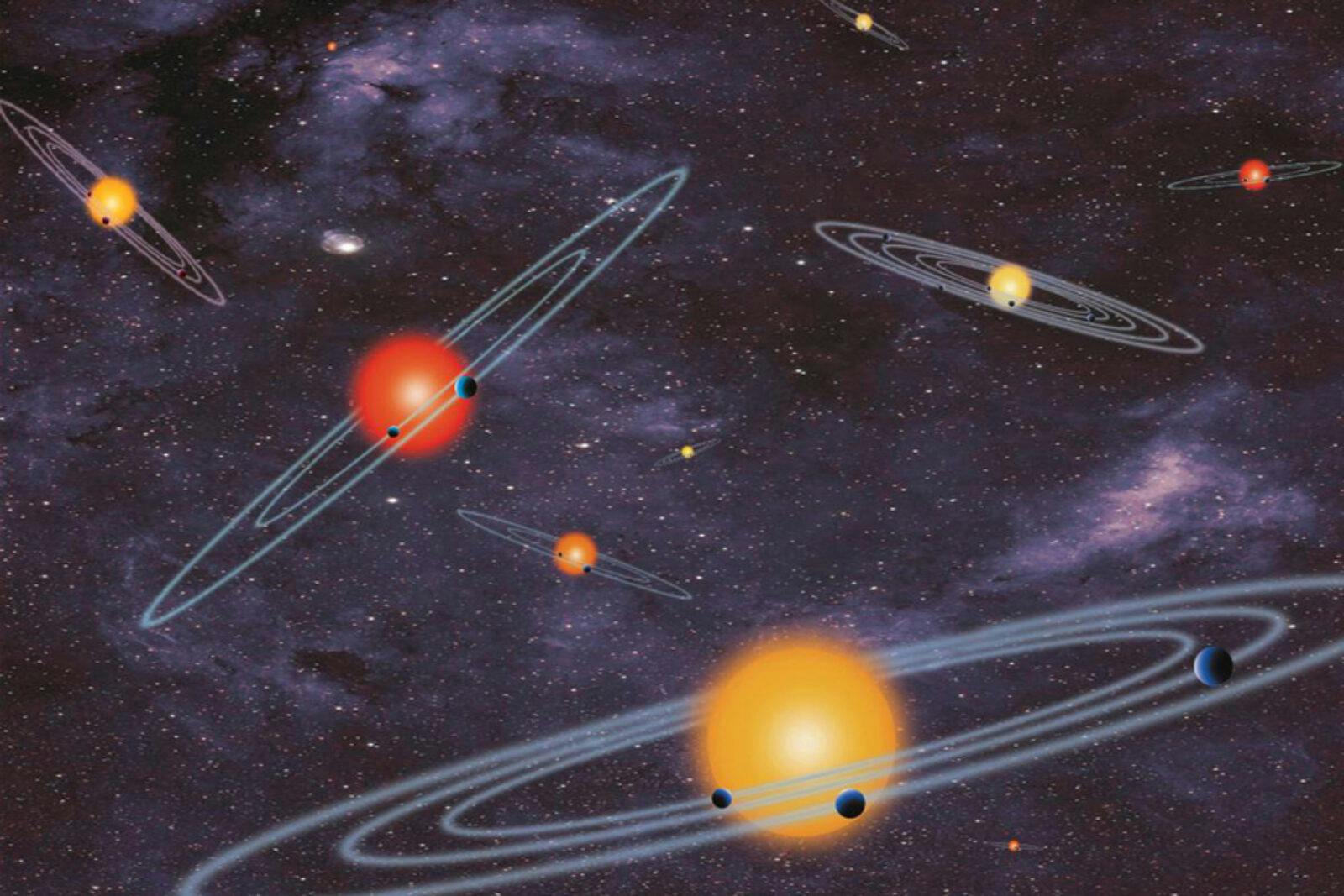
The Author
The scales involved in astronomy never cease to amaze. A single light-year—a ho-hum unit of cosmic measurement, based on how far light travels in a year—is about six trillion miles. The nearest star, Proxima Centauri, is 4.25 of those light years away, or a highly-inconvenient-for-visitation 25 trillion miles. And while we're talking farness, just to go all the way to the max, the observable universe—that is, the spherical realm of the cosmos that can be observed by our telescopes, again based on the speed of light—is about 90 billion light years in diameter. But in backing away from these various "-illions", take a moment to consider neutron stars. These objects are the remnants of colossal stars that once dwarfed the Sun. Neutron stars, however, are only about the diameter of a city—roughly six miles across. It is therefore stunning, even again with all these stonkingly large numbers being thrown around, that astronomers have witnessed the relative cosmic speck of a neutron star pumping out a super-high-energy beam of material that spans 40 trillion miles. That's a distance that amply exceeds the distance between us and Proxima Centauri. It's an impressive astrophysical feat, for sure, and one with fascinating context and consequences, as will be explained further below in our latest roundup on recent news from Kavli astrophysics institutes.
Ixnaying a trio of exoplanets
Since the discovery of the first exoplanet around a Sunlike star in 1995, more than 5,000 of these worlds—along with another 5,000 candidate worlds awaiting further confirmation—have been tallied up by researchers. In an exception to the norm of "ever more," though, researchers at the Massachusetts Institute of Technology Kavli Institute for Astrophysics and Space Research have had to remove three worlds from the overall sum. Over the decades of exoplanet science, stellar science has improved, too, and scientists now can better differentiate between what is a very small star and a very large planet. Three suspected planets, originally spotted by the Kepler spacecraft during its observing run from 2009 to 2013, are now reckoned to be firmly in the former and not in the latter category. The three supposed exoplanets are all larger than twice the size of Jupiter, and thus too big to plausibly not have nuclear fusion occurring in their hot, dense cores, which is in the mark of a star. Culling the exoplanet rolls in this way will help to advance the study of both stars and planets, and the dividing line between them.
Keeping our eyes peeled for the next major supernova in modern times
A story by Nature conveys the profound scientific windfall expected when the next nearby supernova pops off. Someone who is eagerly awaiting this event is Masayuki Nakahata, a principal investigator at the Kavli Institute for the Physics and Mathematics of the Universe (Kavli IPMU) at Tokyo University. He was a graduate student when the last nearby supernova delivered such a windfall back in 1987. Nakahata is now the leader of Super-Kamiokande, the largest neutrino detection experiment of its kind in the world, and which will play a key role in detecting the vanguard neutrinos from the unfolding stellar explosion. By training modern instrumentation on the next proximal supernova, researchers expect to gather critical new data on how heavy elements are forged in the universe, as well as insights into fundamental physics. With any luck, Nakahata and his global colleagues will not have to wait much longer.

A deep look into the dark side of a "hot Jupiter"
It's a tale of two hemispheres for the exoplanet WASP-121b. MKI scientists are part of a team that has revealed key details for the first time about the dark side of this kind of world, known as a "hot Jupiter." These planets orbit scorchingly close to their host stars and are tidally locked, meaning one hemisphere faces the star in perpetual day while the opposite side experiences permanent night. The day sides of hot Jupiters have been observationally characterized before, but night sides, which are ten times dimmer than day sides, have eluded detailed study. Using a camera on the Hubble Space Telescope, researchers were able to deeply characterize both hemispheres, painting a picture of WASP-121b's global climate. The planet evidently has a water cycle, like Earth, but of a vastly different nature: Clouds of water form on the cold night side, but then are pushed by 11,000-mile-per-hour winds onto the day side, where the water atoms break apart into their atomic constituents, only to then get recirculated by the same winds to the night side to form clouds again, and so on. A similar cycle also exotically happens for iron and the mineral corundum (which composes rubies and sapphires).
40-trillion-mile-long beam of matter and antimatter pumped out by diminutive stellar remnant
Researchers at the Kavli Institute for Particle Astrophysics and Cosmology (KIPAC) at Stanford University have helped answer how free-flying antimatter forms and disperses in our Milky Way Galaxy. Antimatter is like normal matter but has the opposite charge. This fundamental difference means that when matter and antimatter meet, they mutually annihilate into pure energy. Because our universe is completely dominated by matter, only very small amounts of antimatter can exist at any given time. Nevertheless, positively charged counterparts to electrons, called positrons, reach Earth at far higher rates than expected. In answering this riddle, the KIPAC researchers have observed a gigantic jet of matter and antimatter, stretching across more than six light-years (around 40 trillion miles), originating from an extreme object known as a pulsar. Pulsars are neutron stars—the ultra-dense remnants of massive stars—that spin multiple times per second, sending out a beam of radiation that sweeps past Earth. Pulsars also generate tremendously strong magnetic fields. The intense conditions form matter and antimatter out of energy, in a reversal of Albert Einstein's famous equation E = mc2 (energy = mass x the speed of light squared). Scientists have previously observed bright high-energy glows around pulsars indicative of just-formed antimatter trapped in the pulsar's magnetic field and annihilating with nearby matter. But in the case of a particular pulsar that is zipping through space (as all cosmic objects do), the Stanford researchers surmised that the stellar remnant caught up to its own bow shock—a compressed area of gas in front of the pulsar, similar to water piled up in front of a fast-moving boat. In so doing, the pulsar's magnetic field and bow shock connected in a way that allowed positrons to "leak" out and reach Earth. This phenomenon, repeated for other pulsars in our galaxy, neatly accounts for the observed antimatter excess.
Most detailed simulation yet of the universe's blooming
A new simulation called Thesan—named after the Etruscan goddess of the dawn—is giving researchers an unprecedentedly detailed look at critical periods of change in the early universe. The simulation models the evolution of a cubic volume of space with sides running 300 million light years in length and temporally covering the era from about 400,000 to a billion years post-Big Bang. Thesan captures the collapse of dark matter into the so-called cosmic web, a structure evidenced to us by all the tremendous amounts of gas that clumped along the dark matter and grew into galaxies we can observe. The stars in the hundreds of thousands of galaxies that emerged in Thesan's simulated expanse emitted radiation that energized ambient gas, transforming it into the ionized state the gas remains in through today. MKI researchers are part of the Thesan project, which successfully brought together precision models of galaxy formation and cosmic dust formation with a new algorithm for the interaction of light with gas. The Thesan results jibe remarkably well with what we think we know about a hard-to-observe cosmic era. New observatories like the James Webb Space Telescope will gather up key new data that might further bear the model out.
