Forward on Thicker Nickelate Superconductors
by Alan S. Brown
Research Highlights from Kavli Nanoscience Institutes
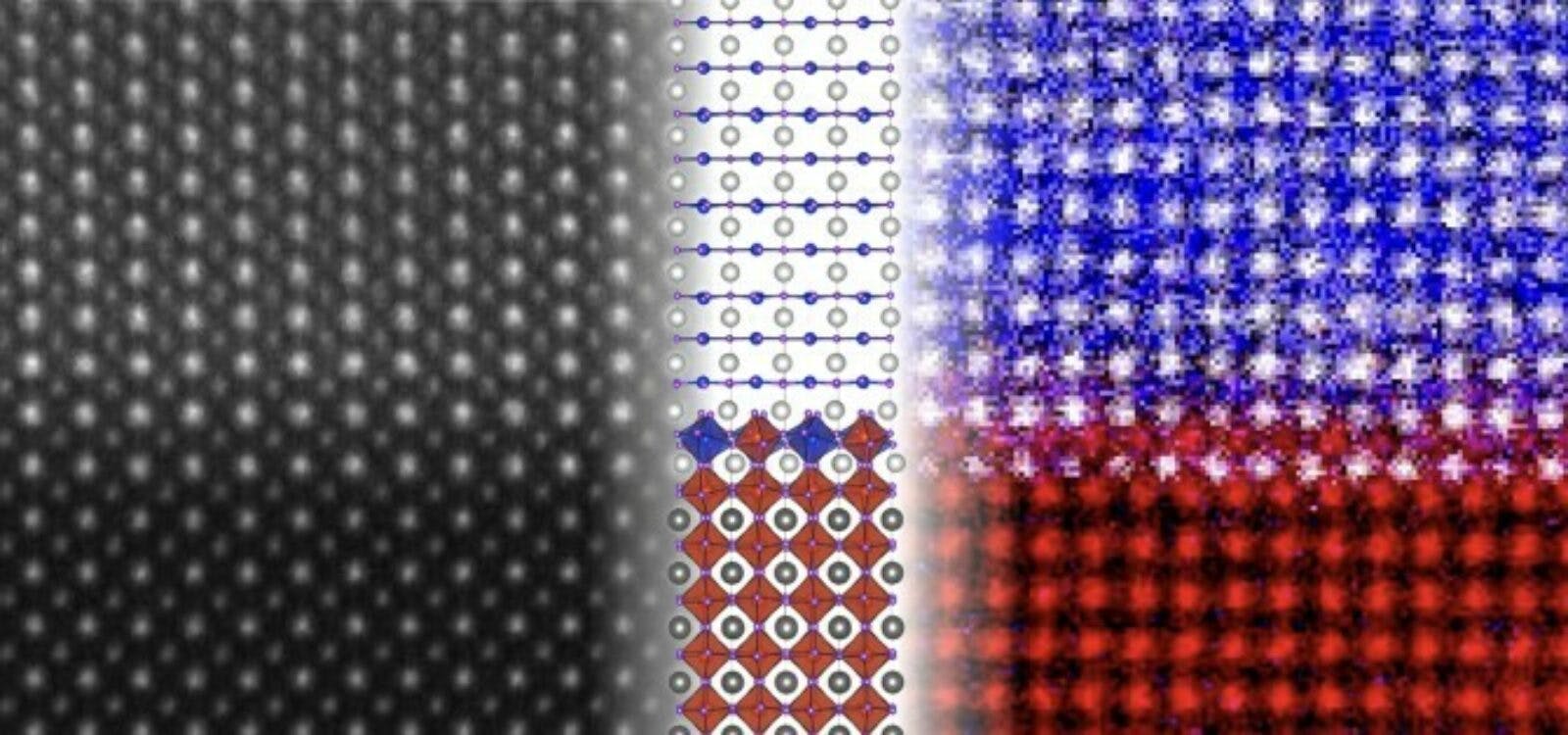
The Author
In this collection of research highlights from Kavli Nanoscience Institutes, we look at heroic efforts to isolate the 36 proteins needed to unzip a strand of DNA to see how they power this process. We also view a video that explains how a newly empowered mass photometry technique gives us a new tool to study protein interactions, and a combination of instruments that shows why we should be able to make thicker nickelate superconductors. We also garner some old school insights, such as a 120-year-old equation that could help us transform greenhouse gases into useful chemicals.
Unzipping DNA, up close and personal
Molecular imaging is changing the way we view the biochemistry of life. One example is the work done to understand CMG, a molecular motor that unzips the DNA double helix so it can be read and copied. This is more difficult than it sounds because it takes 36 individual proteins and a cascade of reactions to assemble and activate CMG. Isolating those reactions among all the biochemical give and take in a cell is almost impossible. Instead, researchers led by Nynke Dekker, a member of the Kavli Institute of Nanoscience at Delft, Ph.D. graduate Daniel Ramirez Montero, and John Diffley of Francis Crick Institute, removed the 36 proteins from the cell and attached fluorescent labels to some of them. Then, while holding a CMG-containing DNA molecule with optical tweezers, they scanned it with a green laser to make movies. This enabled them to visualize how the proteins reconfigured into CMG and how CMG unzipped the DNA as it moved along it. Future studies may uncover new details about the process, teaching us how cells transmit genetic information faithfully and the type of errors that contribute to genetic disorders or cancer.
Closing in on protein dynamics
Advances in measurement instruments spark many breakthroughs in nanoscience. One example is mass photometry, which uses light scattering to learn how proteins interact with one another. The technique basically shines light through a liquid suspension of proteins in a glass capsule. It identifies proteins by how the light they scatter interferes with the light reflected directly back to the light source. A problem with the technique has been that the reflected light washed out the tiny amount of scattered light. In 2018, a team led by Phillip Kukura, a member of the Kavli Institute for Nanoscience Discovery at Oxford, found a way to reduce the reflected light so protein light scattering stood out more clearly. This has enabled researchers like Manish Kushwah, a post-doctoral fellow in Kukura’s group, to study how proteins interact with one another. One example is the spike of Covid-19, which latches onto ACE2, a human cell protein. Kushwah wants to understand the structure of the spike, how it binds to other spike proteins, and the strength of its attachment of ACE2. By understanding how proteins interact with one another, Kushwah hopes to provide clues for medications to defeat the coronavirus. The latest video from Oxford describes his efforts.
Old equation equals new CO2 insights
One way to remove excess carbon dioxide from the atmosphere is to transform it into something useful. That is why some researchers are looking for ways to reduce CO2 into ethane, ethanol, or the chemical building block ethylene, which is used to make a wide range of plastics. A problem, however, is that those processes also yield difficult-to-remove byproducts that make those reactions uneconomical in the real world. What engineers need is a better way to predict what variables will push reactions to make more ethylene. They may have found their answer in the 120-year-old Cottrell equation, which estimates the chemical products generated by reactants based on the current they generate. Cornell Ph.D. student Rileigh Casebolt DiDomenico learned about the equation while taking a class from Héctor Abruña, a member of the Kavli Institute at Cornell. Together, the two and Tobias Hanrath tested the equation against lab experiments and found it made excellent predictions with few assumptions. This made it a valuable addition to the toolbox for exploring chemical routes to CO2 reduction, in addition to computer models, which require more assumptions and greater computational overhead.
Forward on thicker nickelate superconductors
Although theorists predicted superconductivity in nickel-based oxides for 20 years, no one had synthesized working sample until 2019. Even then, they could only get the material to work when they were less than 20 nanometers thick layered over a support substrate. This led some researchers to conclude that superconductivity arose from the interface created by the nickelate and strontium titanate support structure. Lena Kourkoutis, a member of the Kavli Institute at Cornell teamed with Ph.D. candidate Berit Goodge to discover whether this was true. They used an advanced scanning transmission electron microscope and electron energy loss spectroscopy to probe the interface between the nickelate and strontium titanate. The interface they found was unpredicted and, more importantly, has a structure that prevents the type of electronic charge buildup needed to achieve superconductivity. This means nickelate is almost certainly the superconductor and that future research should pursue ways to make larger nickelate crystals.