Frontiers in Nanoscience
Nanoscience has become a new way of exploring conventional sciences, ranging from chemistry and physics to biology and engineering.
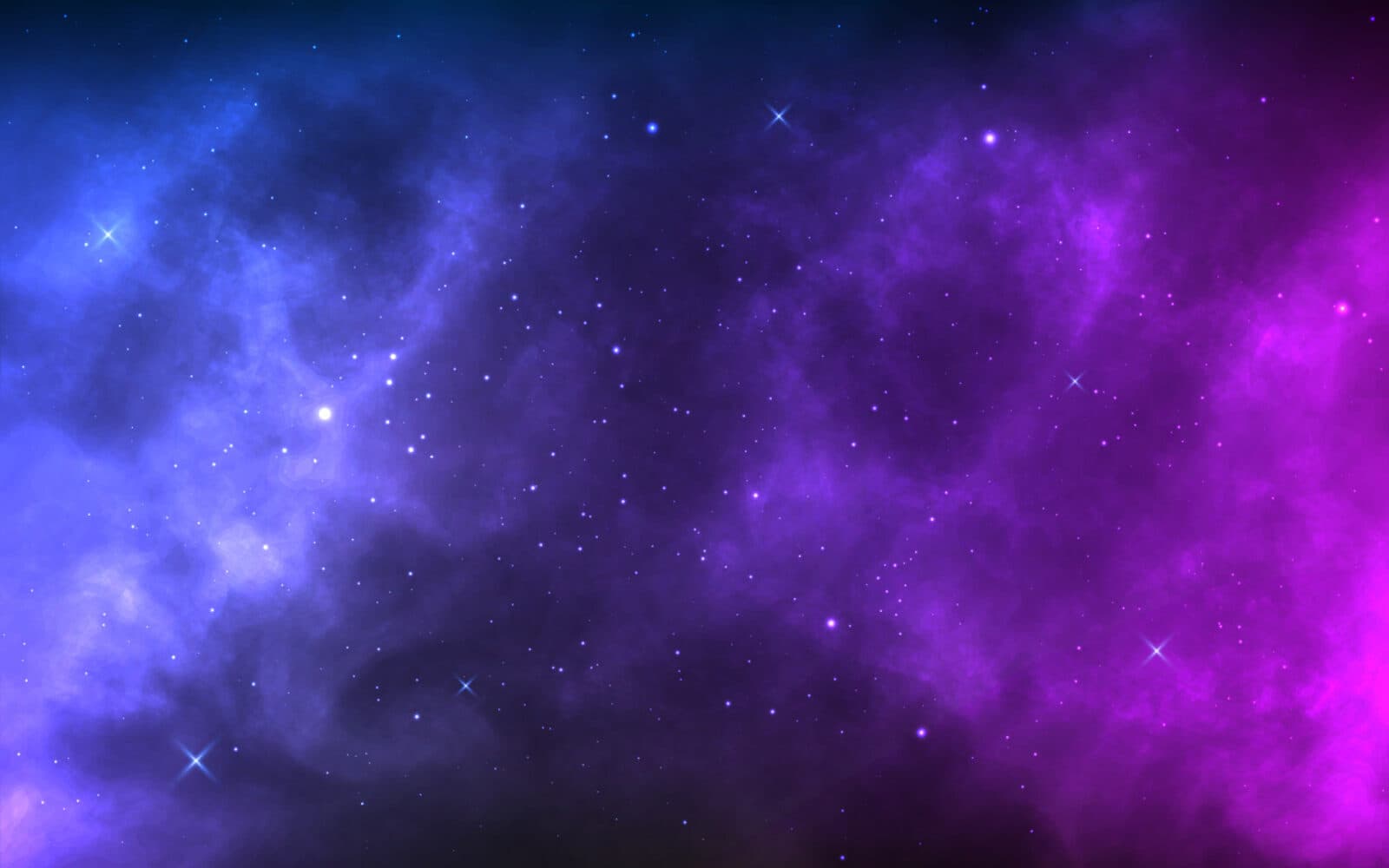
Nanoscience and nanotechnology have captured the public imagination, but they are desperately hard to pin down. They seem to embrace everything from biomedicine to rocket science and computer technology. Some futurologists forecast that nanotechnology could bring doom to humankind, while others that it could be our savior. According to some accounts its impact won’t really be felt for decades; others point out that it has reached the marketplace already. In movies and novels such as Spiderman II and Prey, nanotechnology serves as a deus ex machina for whatever technological plot device is required, in contrast to which the commonly known consumer applications that today boast of their use of nanotech seem almost bathetic: sun creams and stain-resistant trousers.
So what’s the real deal? What are the nanoscientists up to, and should we fear or welcome it?
The Big Impact of the Nano World
Perhaps the one thing anyone who has heard of nanotechnology knows is that it deals with very small stuff. To most scientists, nanoscience and nanotechnology are about doing science and technology with objects that measure no more than a few hundred nanometers in one or more dimensions: very thin sheets or fibers, or tiny blobs of material. This is the “nanoscale”: a nanometer is a millionth of a millimeter, and it’s about the size of some big single molecules such as the protein enzymes in our bodies. A red blood cell is about six thousand nanometers across and a human hair typically 20,000-100,000 nanometers. So yes, nanotech works with small stuff indeed, generally invisible to the naked eye.
In the 21st century, scientists will not only use molecules as building blocks for creating vital new technologies, but possibly as the basis for creating synthetic life.
As you might imagine, it is very hard to get a grip on things this tiny to mold or cut them into shape, then put them where we want them. So what’s the point of making stuff at such a Lilliputian scale? There are several answers, depending on your aims. In computer science and information technology, the incredible advances in computer power over the past few decades have come largely from making the transistors of microelectronic circuits ever smaller. Now millions of them can be carved on a single silicon chip. We can have more computing power in our kettles than was on board the first Apollo missions. Using nanotechnology, the speed and power of computers will continue to increase. In fact, nanotech may supply the only means for the computer industry to continue meeting the public expectation that next year’s machines will be smaller and lighter while being even brainier.
One of the benefits of nanoscience in medicine is that it lets us make interventions at the natural size scale of living cells themselves, rather than trying to affect what these cells do using clumsy instruments that are much bigger. Nanoparticles – lumps of material just a few nanometers wide – can be attached to proteins or other molecules to watch how they move around the cell; for example, acting as labels that glow when light is shone onto them. These particles and other nanoscale structures can be designed to pass through the bloodstream and latch onto invaders ranging from viruses to malignant cells, such as those that cause cancer, then perhaps releasing drugs or heat to kill them. And sensors that use nanotechnology could detect substances in our bodies at far smaller concentrations than is currently possible – maybe as tiny implants offering a continuous, highly sensitive readout of our state of health.
Nanotechnology might allow us to design the properties of new materials from the bottom up; for example, making super-strong fibers or materials that adapt to their environment or when damaged heal themselves. It promises better and cheaper ways of harvesting sunlight for energy, or detecting and eliminating pollutants in water and air. It seems that just about every field of science stands to gain something from working at the nanoscale. Ultimately, a mastery of matter that lets us build things at the scale of atoms and molecules means, in principle, we can do it more efficiently and cleanly, with less waste of materials and energy, And it means we can measure things more sensitively, design properties and functions with more versatility, and start mimicking some of the clever things that nature achieves with her own “natural nanotechnology.”
How We Began Moving Atoms
All this promise has appeared on the technological horizon within the past two decades or so. Although the word “nanotechnology” was coined in 1974, it didn’t mean very much until, the following decade, the tools were invented that made it possible to look into and manipulate the nanoworld. As early as 1959 the physicist Richard Feynman described a visionary goal of moving atoms about one by one. He had no idea how to do it or even if it would ever be possible; but in the early 1980s, scientists at IBM’s laboratories in Zürich, Switzerland devised an instrument called the scanning tunneling microscope (STM). This let researchers “see” atoms individually.

The STM and related microscopes are one of the key enabling technologies that have let nanotech flourish. But making nanoscale structures atom-by-atom this way is a very slow process. One solution, also developed at IBM Zürich, is using many needle tips to manipulate the material. The so-called “Millipede” is an array of thousands of such tips – the latest version has 4000 of them – that work in parallel to modify a material at the nanoscale. Each tip is individually controllable and the plan is to use them to write information into plastic films. This will be done much as it is for today’s CDs and DVDs – by creating little bumps on the surface – but at much smaller scales so that more information can be stored in the same amount of space. The Millipede tips don’t move single atoms; they create little indentations where they have been heated up to melt the plastic. Each dimple is 10 nm across, so that 200 billion of them can be stored in a square inch of plastic film, like a kind of miniaturized Braille.
Packing Information with Molecules
This is a reminder that information technology is one of the biggest driving forces behind nanotechnology. With data packed more densely, you can start to imagine DVDs containing whole libraries of movies, or digital cameras that never run out of space to hold snapshots. Researchers in California have used nanotechnology to make a memory device that packs 160,000 memory elements into a space no bigger than the surface of a white blood cell, and the information isn’t held in switches made from silicon, as in normal silicon chips, but in switchable molecules. Designed by chemist Fraser Stoddart, who holds the Fred Kavli Chair in Nanosystems Science at the University of California, Los Angeles, and his co-workers, these molecules are called “rotaxanes” because they are shaped like wheels or hoops threaded on an axle. Each axle holds a single hoop, which can shuttle between two different positions when a voltage is applied to the molecule. This shuttling triggers a change in the electrical current that passes through the molecule from end-to-end. That’s just like the switching of a transistor, which allows binary information – a string of 1’s and 0’s, corresponding to the switch being “on” or “off” – to be recorded in an array


The Promise of Nanotubes

Information technology isn’t just about big memories. Computer circuits also have to be able to process information, which again they do today using transistors carved from silicon. But the current carving techniques can’t easily make devices smaller than about 100 nm or so, which is why some researchers think that nanotechnology could enable further miniaturization by letting the devices be built from the bottom up – from atoms and molecules – instead of making them by top-down engraving of silicon.
One of the most promising approaches is to make transistors from objects called carbon nanotubes, which are tubes of pure carbon just a few nanometres across, with walls one atom thick. Each nanotube is basically a single tubular molecule, and some of them are semiconducting, like silicon – which means they can be used to make electronic devices such as transistors that are much narrower than those currently made from silicon (Figure 3). The challenge now is to ensure that nanotube transistors are reliable and robust enough for use in commercial circuits, and to arrange them just how we want them.

Carbon nanotubes are also remarkably stiff and strong – in effect, they are the ultimate carbon fiber. They are already being used as stiffeners, replacing conventional carbon fibers in products such as tennis rackets and golf clubs, although they remain expensive to make. And they could supply the ideal “girders” for building nanoscale structures and machines: for example, nanotubes with walls made of several concentric layers can act as sleeve-and-axle bearings for rotors, or as extendable nanoscale arms that will lengthen like telescopes. Ultrafine needles made from nanotubes can puncture cell walls without damaging them to deliver drugs (Figure 4).
Illuminating Molecules
Medical science is expected to be another of the main beneficiaries of nanoscience and nanotechnology. Nanoparticles have been developed by Chad Mirkin and his colleagues at Northwestern University in Illinois to serve in highly sensitive detection systems for particular kinds of DNA or proteins – for example, to look for specific genes in a patient’s blood. The metal nanoparticles have surfaces coated with molecules that latch onto the biomolecules being studied, which binds the nanoparticles into clusters. These clusters scatter light, rather like the grains of silver in an exposed photographic film, and so a solution of nanoparticles changes color or darkens, offering a quick and convenient “readout” for diagnosing the presence of the biomolecules. The Northwestern researchers have used this method to produce commercially available biosensor devices.

Nanoscience pioneer Jim Heath, a member of the Kavli Nanoscience Institute at the California Institute of Technology, believes that arrays of nanowires like those used in the “crossbar” memory device above can also serve as highly sensitive biosensors, since their electrical conductivity may change when biomolecules become stuck to their surface. These arrays can be made small enough to examine the contents of individual cells, and they might detect substances such as proteins that signal cancer at far lower concentrations than is currently possible. This would allow incipient cancers to be detected much sooner, making their treatment more likely to succeed.
Some of the tools that have been developed for manipulating and investigating objects at the nanoscale have become immensely valuable for studying the “nanomechanics” of living systems. The atomic force microscope, a relative of the STM in which a needle tip is used to measure forces acting at the nano- and molecular scales, has been used to study how individual biomolecules change shape – for example, when a single protein (a chainlike molecule) is pulled out from its normal, crumpled-up shape into a stretched-out strand. This can provide understanding of how proteins take on the highly specific shapes that are crucial to their biological function, or how biomaterials made from proteins, such as elastic tissues, muscle or silk, achieve their resilience. Another valuable tool for biomolecular nanoscience is a technique called laser trapping (or optical tweezers), in which nanoscale objects are “held” by intense, finely focused laser beams. Because of an interaction between the object’s electron clouds and the strong electromagnetic field of the light, the object gets pulled towards the brightest part of the laser spot. This supplies a way of dragging molecules and nanoparticles around, and has even been used to tie a knot in the long-chain protein actin by using optical tweezers to tug on two microscopic beads at each end (Figure 5).
Unraveling the Fabric of Life
One of the most dramatic conjunctions of nanoscience and biology comes from the realization that the fabric of life – DNA – is also an ideal material for nanoengineers. DNA is a long, chainlike molecule that encodes genetic information in its chemical structure, and this enables two molecular strands to stick together in the famous double helix – the sticking secure only if the information on one strand “matches” that on the other. So techniques for making DNA artificially, with arbitrary sequences of information in a strand, can be used to program specific strands to stick together in complex shapes. Using this idea, researchers have made nanoscale objects such as cubes and other geometric cages from DNA, as well as grids and ladders. Paul Rothemund at the California Institute of Technology has invented a scheme for programming DNA strands to assemble themselves into just about any flat shape you like, from smiley faces to maps of the world, all measuring just a few nanometers across (Figure 6). DNA tags could also be attached to other nanoscale objects so that they can assemble themselves into organized structures such as electronic circuits.

This shows how nanotechnology can profit from exploiting some of the tricks that biology uses for making living organisms – in particular, using self-assembly of “programmed” molecules to create structures that are far more complicated than those we could hope to make “by hand.” These tricks might ultimately provide nanotechnological devices and structures that make copies of themselves (replicate), pass on information and perhaps qualify as genuinely living things.
This might sound alarming, and it’s true that such possibilities carry dangers and ethical responsibilities, along with great promise. At this stage, however, the principal potential dangers of nanotechnology hinge more on the question of whether nanoparticles could pose health risks if they get into the body. Their chemical behavior can be quite different from that of larger chunks of the same substance, so we can’t assume that what’s non-toxic at the everyday scale remains safe at the nanoscale. We don’t yet know enough about such risks, or about how nanoparticles might be transported around the body or the environment. This is now an active area of nanotech research and it’s a priority to find out more, although the initial indications are there is no urgent cause of alarm about nanoparticle toxicity.
Like any new technology, nanotech offers both benefits and risks. But the balance probably depends much more on how we elect to use it, than on anything intrinsic to the technology itself. The choice, in other words, is ours.
Further reading
- M. Ratner & D. Ratner, Nanotechnology: A Gentle Introduction to the Next Big Idea. Prentice Hall, 2003.
- R. A. L. Jones, Soft Machines: Nanotechnology and Life. Oxford University Press, 2004.
- Scientific American special issue on nanotechnology, July 2004.
- Understanding Nanotechnology – a collection of articles from Scientific American. Warner, 2002.