Mapping the Brain’s Cell Types and Connections
by Sally Johnson
Understanding how the brain’s different cell types communicate may reveal how neural circuits work
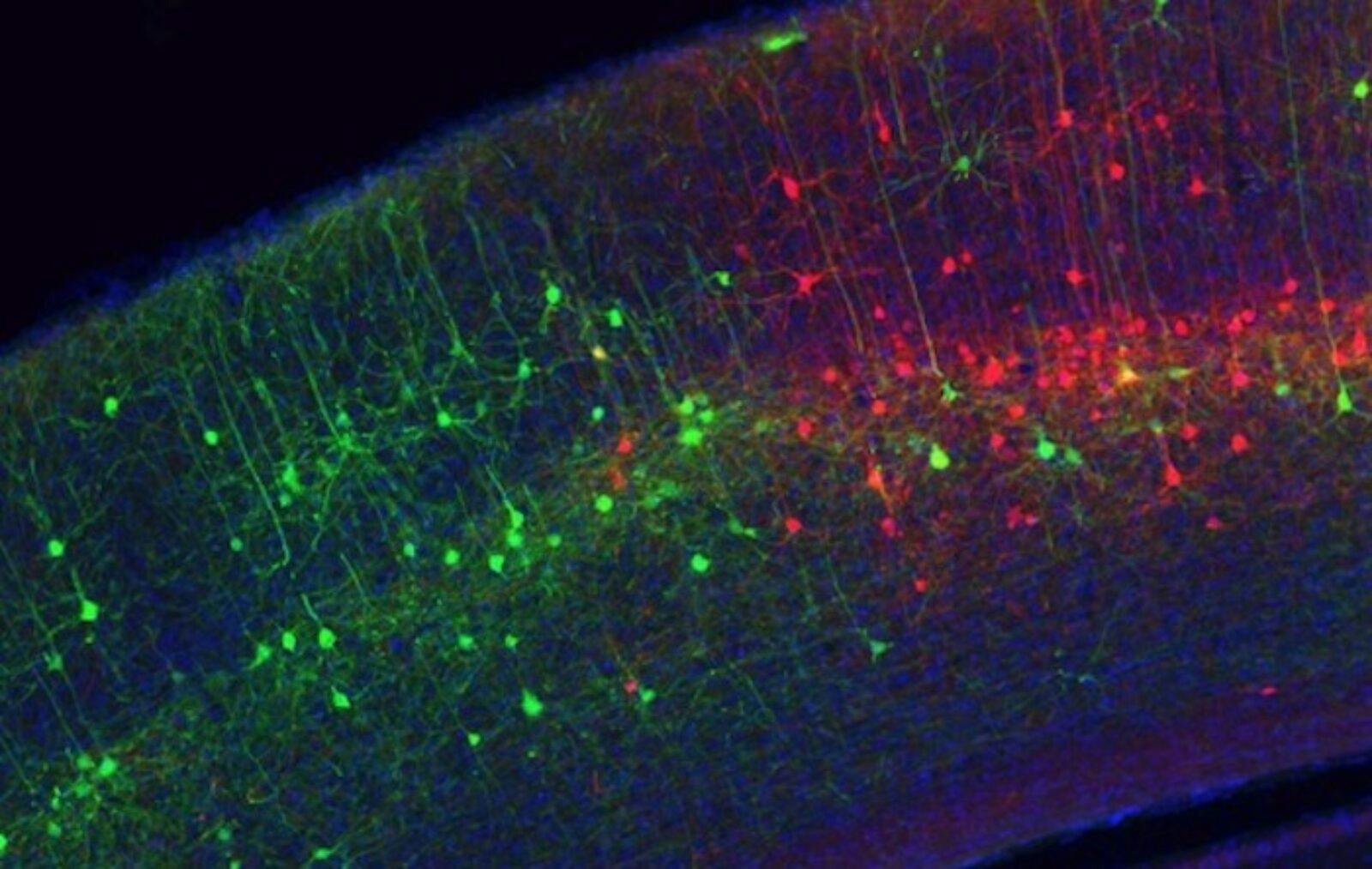
The Author
The Researcher
The human brain is the most complex and mysterious biological system, with many of its billions of cells firing simultaneously in intricate patterns and networks. By exploring how each of the brain’s neurons and cell types are connected and what their functions are, Edward Callaway, co-director of the Kavli Institute for Brain and Mind, as well as the Vincent J. Coates chair in Molecular Neurobiology and professor in the Systems Neurobiology Laboratory at the Salk Institute, and colleagues are creating a neural map to better understand how neural circuits work.
“Brains are complicated, but it’s clear that proper brain function and health relies on very tight regulation of biological mechanisms—genes and their expression, cellular health and function, regulation of synaptic weights between neurons, interactions between ensembles of hundreds of thousands of neurons—and regulation through neuromodulatory systems [small pools of neurons that can affect cognitive behavior],” he says. “It’s really quite astounding and a testament to the power of evolution that these nervous system machines we all have in our heads work as well as they do as often as they do.”
If you want to figure out how a system works, one of the first things you need is a parts list.
Edward Callaway, co-director of the Kavli Institute for Brain and Mind
During the past 25 years, Callaway’s lab has explored the various cell types and their connections within the cerebral cortex—the outermost layer or the bulk of the brain. In humans, he describes the cerebral cortex as resembling “a folded up pizza put inside your skull.”
Humans and mice have similar cerebral cortexes in that they possess many of the same cell types that are connected through the same kind of wiring rules. Presumably they also have similar functions, so studying mice and other animals can help decipher how human brains work.
Advances in technologies to study cell types and connections have improved dramatically within the past few decades. “For example, we once thought there might be a handful of cell types that we could distinguish from their different morphologies and stains for a few different antibodies,” Callaway explains. “And we had to painstakingly try to decipher their connectivity with recordings from small numbers of cells. But now we know that there are hundreds of cell types and we have much better ways of identifying their connections.”
Using mouse lines to target those cell types combined with molecular, genetic, and viral tools for interrogating connectivity and function has enabled important progress in determining their interactions and functional roles.
Back in 2007, Callaway developed a tool that uses a modified rabies virus to trace single connections between neurons. “If you want to figure out how a system works, one of the first things you need is a parts list,” he says. “Then you need a diagram of how they interact and work together, so you can make theories about how it works. This is how we view cell types: knowing how cell types interact is sort of a wiring diagram, which we need at the resolution of cell types.”
His lab was able to take advantage of the ability to already target gene expression of specific cell types within the brain, and built upon it by making modified rabies viruses that collectively infect a specific cell type and then spread to and label only the neurons that make direct connections to what he calls “starter cells” across the whole brain. These methods are now used by researchers around the world to create a wiring diagram across the whole brain describing the inputs to various cell types.
“Our approach is being used for everything from understanding the circuits responsible for neuromodulatory systems, which are probably involved in depression and anxiety, to interactions between cell types that regulate cognitive function within the cortex, which are likely related to neural circuit diseases,” Callaway adds.
Now, he says tools are desperately needed to genetically access far more of the hundreds of cell types to identify connectivity and function at higher levels of resolution.
So his lab is working with other labs (such as Bing Ren’s Laboratory of Gene Regulation at UCSD, and Joe Ecker’s Genomic Analysis Laboratory at Salk) that generate epigenetic data to identify specific enhancer candidates to make artificial viral vectors that can be injected into a brain to express any gene they want within a specific cell type.
“We hope that the enhancers will provide the needed genetic access to presently inaccessible cell types,” Callaway says. “The field will be able to use these tools to dramatically increase our understanding of neural circuit function. And these enhancers will be able to be used in gene therapy, one day down the road, decades from now.”
There is a broad range of research going on within the mind and brain research community in San Diego, and exciting discoveries are made all the time. “I’m most familiar with the work on neural circuit mechanisms that underlie perception and behavior in animals,” Callaway notes. “But to have an impact on health and disease, this work must be informed by less invasive studies of human patients and volunteers. A question now is: how can we use our knowledge of how cell types contribute to function within mice to identify mechanisms that give rise to neurodevelopmental and behavioral disorders in people?”