Quantum Vibrational Energy
by Alan S. Brown
Research highlights from Kavli Nanoscience Institutes
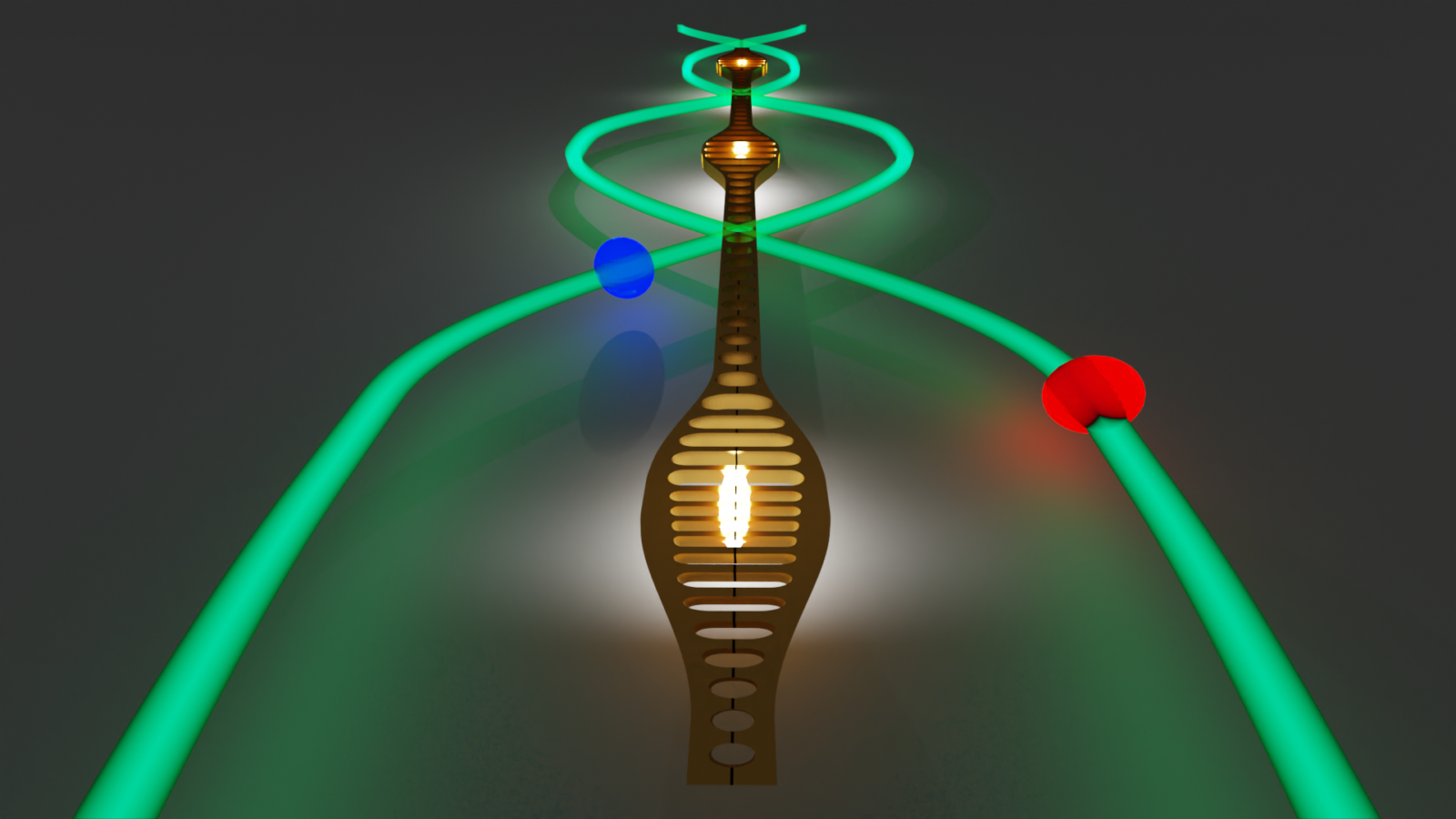
The Author
The Kavli Nanoscience Institutes end the year with several exciting advances. One, from the University of Oxford, involves a new model to help predict the severity of Alzheimer’s disease in individual patients and investigate why some people are more resilient than others. The second, from Cornell University, illuminates what noncoding RNA – the “dark matter” of cells – is actually doing there. Also from Cornell is a method to measure heat transfer in the smallest particles. Delft Technology University researchers developed a device that might allow us to entangle qubits generated by very different technologies. And finally, from the University of California, Berkeley, we have the generation of a catalyst that converts compounds made by artificial photosynthesis into useful chemicals.
Predicting Alzheimer’s disease
Researchers know that genetic mutations in the amyloid precursor protein (APP) and the enzymes that break down APP have something to do with Alzheimer’s disease. Yet, although amyloid-related chemistry predicts clinical outcomes, it does not indicate how severe those conditions will become. In fact, some people with extensive amyloid pathology show little clinical impairment while others will little pathology show significant dementia. To link the two, researchers need to go beyond the transgenic rats they now use to study Alzheimer’s. A collaboration involving Richard Wade-Martins, a member of Oxford's Kavli Institute for Nanoscience Discovery, gets us closer to that model. Starting with blood samples from 14 Alzheimer’s patients, Wade-Martins’ team converted the blood cells into stem cells and then coaxed the stem cells to grow into specialized brain cells: neurons. Each neuron reflected unique aspects of an individual patient’s biological characteristics. The researchers then induced damage where the neurons connect to each other at synapses, using amyloid-beta, a toxic protein involved in Alzheimer’s. Their surprising discovery: The greater the synapse loss in the petri dish, the more impaired the actual patient is under observation. The researchers believe this model will help them better understand why some people resist the ravages of Alzheimer’s more than others.
Seeing RNA “dark matter” for the first time.
A simple technique developed in the lab of Kavli Institute at Cornell for Nanoscale Science researcher Iwijn De Vlaminck and his doctoral student David McKellar promises fast improvement to our understanding of how cells work. It focuses on RNA, complements of DNA segments often used to make proteins. This mostly gets done by messenger RNA in the nucleus, where it is tagged with a polyadenylated (poly-A) tail. To understand what is going on in a cell, researchers freeze cells and trap those tails on a chip and count which RNA are located where. Unfortunately, not all RNA is messenger RNA. Noncoding RNAs never get a poly-A tail, so it is hard (if not impossible) to work out what they do in the cell. That’s why McKellar calls them the “dark matter of the transcriptome.” His solution: Inject an enzyme, poly-A polymerase, into the cell. It tags every RNA with a poly-A tail, extending the length of poly-A tails on messenger RNA and giving noncoding RNA a tail so we can study it using the same techniques now used to study messenger RNA. This made it possible to study the elusive role of noncoding RNA in muscle regeneration. There are hundreds or thousands more noncoding RNA in the cell. Because of its simplicity, De Vlaminck expects other laboratories to adapt the technique very quickly.
Mix-and-match quantum processing
Creating conventional computing devices presents many challenges, but getting them to pass information back and forth with one another is not one of them. This is because all electronics use electrons to communicate. This is not true for quantum devices. They rely on entanglement to share information, and there is no easy way to entangle superconducting qubits (an entangled bit of quantum information) used for computing with fermion qubits used to store information or photon qubits used to transmit it. Simon Gröblacher’s lab at Kavli Institute of Nanoscience at Delft Technology University hopes to hurdle that barrier. His group focuses on phonons, units of quantum vibrational energy, that can entangle qubits derived from light, electrons, and superconductors. The problem has been confining those phonons to a chip, something Gröblacher’s team has managed to now do using waveguides. They have already demonstrated that they can guide single phonons along a specific path. Next, they plan to use a beam splitter to route phonons onto different paths and a phase shifter to change their state while traveling. The technology is scalable to handle lots of phonons and could be used to conduct complex quantum experiments and perhaps form the basis of a new type of quantum connector.
Measuring nanoscale heat movement
If you want to know how heat moves through a slab of metal, just put a thermometer at either end and record their temperatures. Now, imagine what would happen if the thermometer was hundreds of times larger than that slab of metal. Its very size would make it too inaccurate to measure that small distance. This is exactly the problem with measuring thermal conductivity and diffusivity in nanoscale objects. Whether it is a sliver of semiconductor or a protein, even the smallest calorimeter probes are too large to do the job. One solution is optical wireless integrated circuits (OWiCs)from the lab of Paul McEuen, a member (and former co-director) of the Kavli Institute at Cornell. OWiCs are tiny, nanofabricated chips that contain all the components needed to make optical measurements without contact. The chips, only 100 micrometers in size, can measure samples down to 100 nanograms within microseconds. That’s good enough to investigate everything from aerogels formation to superconducting phase transitions to the binding mechanisms of amino acids. To prove it, the researchers measured thermodynamic phase transitions in a well characterized liquid crystal 4-cyano-4’-pentylbiphenyl, or 5CB, and gadolinium.
Copying nature’s route to chemicals
For decades, we have known that bacteria use sunlight-generated electrons to break carbon dioxide and water into two asymmetric chemical groups, carbonyl (CO) and methyl (CH3). Bacterial enzymes then reassemble the two into acetate, a chemical researchers hope to upgrade into fuel, drugs, or other chemicals. Peidong Yang, director of the Kavli Energy NanoScience Institute at UC, Berkeley, has now developed a bacteria-inspired catalyst that also produces acetate. When placed under an electrical bias and exposed to CO gas and liquid methyl iodide, the copper-silver nanoparticle catalyst combines the CH3 and CO groups to form acetate as well as ethanol and acetone. The side-by-side silver and copper nanoparticles worked together to turn turned C02 into CO and CH3I into CH3 and then joined the two to form acetate. Yang believes the research could lead to more efficient chemical and fuel plants based on artificial photosynthesis.