Strange States of Matter
by Alan S. Brown
Research Highlights from Kavli Nanoscience Institutes
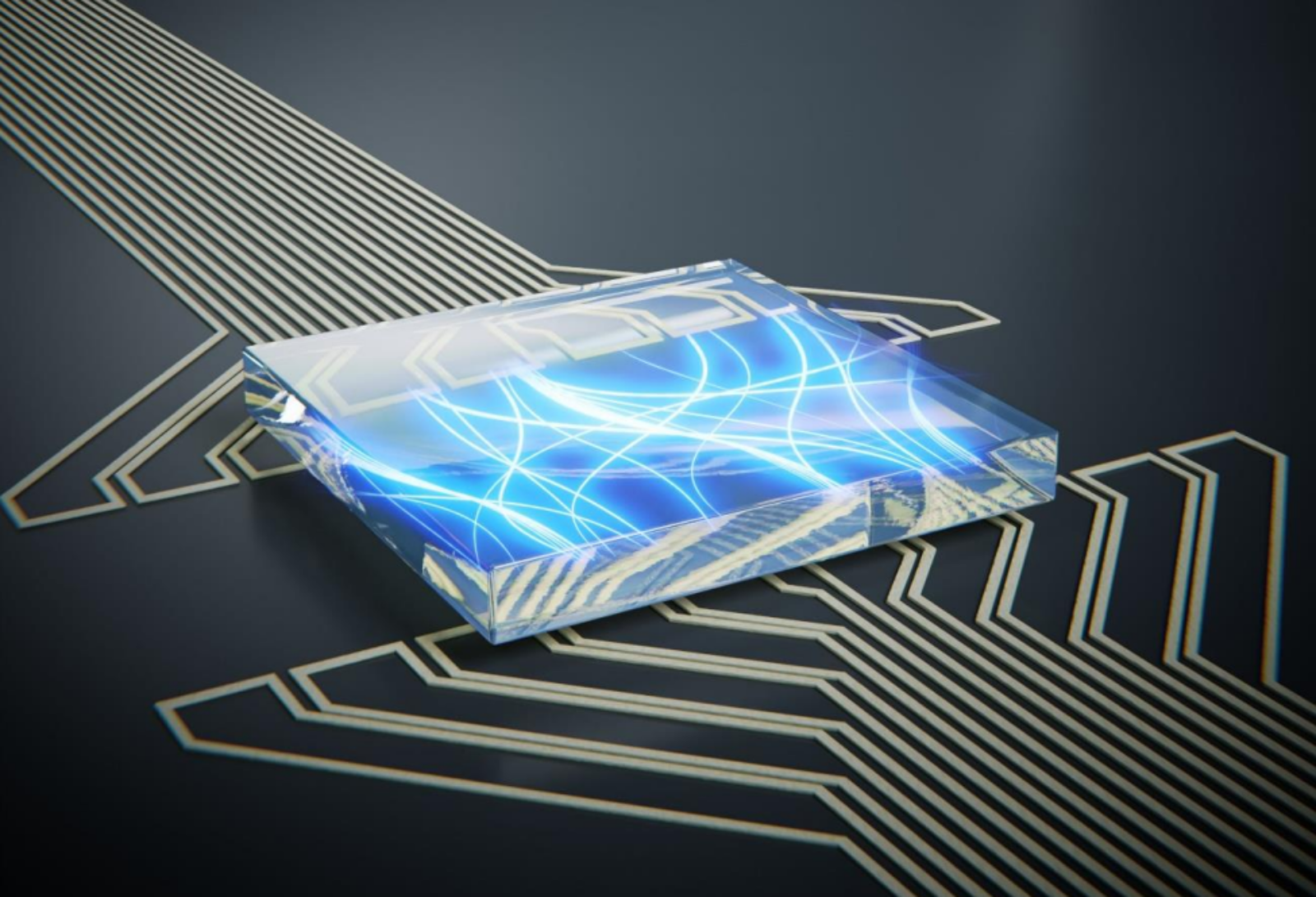
The Author
For decades, computers grew cheaper and more powerful. The reason was Moore’s Law, an observation that the number of transistors doubled every two years. For that to happen, features had to shrink from tens of micrometers sizes to tens and then single-digit nanometer size. The smaller the transistors, the faster they operated and the more companies like Intel could squeeze on a wafer (which lowered the cost). Unfortunately, semiconductor features have been shrinking more slowly, and the cost of new factories to make them have offset cost savings. The race for faster computers is one reason researchers have embraced quantum computing. This month, we look at some of the research that might enable this technology. This ranges from ways to overcome quantum computing errors to harnessing spin waves and leveraging strange new materials. We also check in with new ways to understand bacterial proteins and why flaws can actually improve the strength of 3D printed parts.
A random solution for quantum computing

In the digital world, the term “random compiling” sounds like a bad thing. After all, devices are either on or off and there is nothing random about it. But in the quantum world, it turns out that random compiling might hold the key to dealing with quantum computing errors that become too numerous to manage as the number of quantum processors grows. The main source of those errors is decoherence, which occurs when qubits (packets of quantum information) interact with their environment and become corrupted. There are workarounds when working with a dozen qubits at a time, but they increasingly fail as the number of qubits grows. Instead of trying to fix or correct coherent errors, random compiling takes a statistical approach to the problem. It generates a series of many logically equivalent quantum circuits, then measures and combines their results to average out the impact that coherent errors can have on any single quantum circuit. The work was done by Akel Hashim, a graduate student in the lab of Irfan Siddiqi, a member of the Kavli Energy Nanoscience Institute at UC Berkeley.
Imaging hidden spin waves
Spin waves are one of many possible routes to quantum computing. They start with ferromagnetic materials whose magnetic moments (strength and orientation) have been aligned. But perturb one of these spins and the others around it will begin rotating in a like manner, creating a spin wave that could carry data—without generating heat. This would slash global demand for energy to run computers. To get there, however, researchers need to first understand how and why spin waves lose their momentum. While existing techniques can image spin waves next to the electrodes and semiconductors used to create them, they cannot see under the electrodes. Toeno van der Sar, a member of the Kavli Institute of Nanoscience at Delft, and his collaborators may have solved that problem with a thin diamond film that includes nitrogen instead of carbon, which creates vacancies in some locations. The defects are extremely sensitive changes in spin wave magnetic fields, which have no trouble penetrating the electrodes. Using this technique, they have discovered how eddy currents in the electrode dampen spin waves. Their goal is to model the system and find ways to make spin waves last long enough to do some good.
Strange states of matter could generate qubits
As we dive into nanospace, the behaviors of materials get wilder and often weirder. The latest example: a two-dimensional topological insulator turns into a quantum anomalous Hall insulator by adjusting the current. It sounds totally esoteric (and it is, if you want to read about it), but theorists have been looking for a material that fits this bill for the past decade. So, why does this matter? Researchers Kin Fai Mak and Jie Shan, both members of the Kavli Institute at Cornell for Nanoscale Science, believe they can couple this material with superconductors to generate qubits, the quantum equivalent of a computer bit, with a conductor that recirculates power without dissipating it.
Straightening out protein modifications
If you have ever seen a model of a protein, they you should instinctively understand why Britain’s Biotechnology and Biological Sciences Research Council has awarded Justin Benesch, a member of Oxford University’s Kavli Institute for NanoScience Discovery, a $7.5 million grant to unravel how subtle modifications drastically alter the function of bacterial proteins. Proteins are convoluted molecules whose shapes matter just as much as their chemical makeup. Something seemingly simple, like adding a single phosphate group, can totally change a protein’s behavior. Existing technology does a poor job of detecting those changes—and showing us how we can better combat infection and antimicrobial resistance. To solve this problem, Benesch is working with fellow Kavli researcher Philipp Kukura and others at Oxford, University of Liverpool, and the Wellcome Sanger Institute to combine three technologies: nanopore, electrometry and mass photometry. All three provide insight into biomolecules. Together, they could enable researchers to study even unstable and difficult-to-detect protein changes.
When bad is good
3D printing metal is one of the world’s most exacting production processes, especially when it comes to aerospace parts. That’s because printing metal by fusing layer after fine layer of metal powders often creates voids where stress can concentrate and initiate cracking or delamination. That is bad news, especially for parts that keep airplanes aloft. But it turns out that some flaws may actually make 3D printed metals stronger, according to Kavli Institute at Cornell member Atieh Moridi and Kavli graduate fellow Jennifer Bustillos. They demonstrated it by making a “lousy,” pore-filled printed part of titanium-aluminum-vanadium (Ti-6Al-4V), a common aerospace material. Then they placed the printed part under extreme heat and pressure using a technique called hot isostatic pressing (HIP). This collapsed the pores, which was good. Even better, it created a dual microstructure where one component reinforced the other to improve strength. It also broke up the directionality of the 3D part’s crystals, giving them the same properties in all directions and making them less prone to cracking. The researchers are now working on reducing the fatigue strength of the final part.
