Unraveling the Quantum Mysteries of Photosynthesis
by Alan S. Brown
Graham Fleming searches for answers in one of nature's "messiest" systems
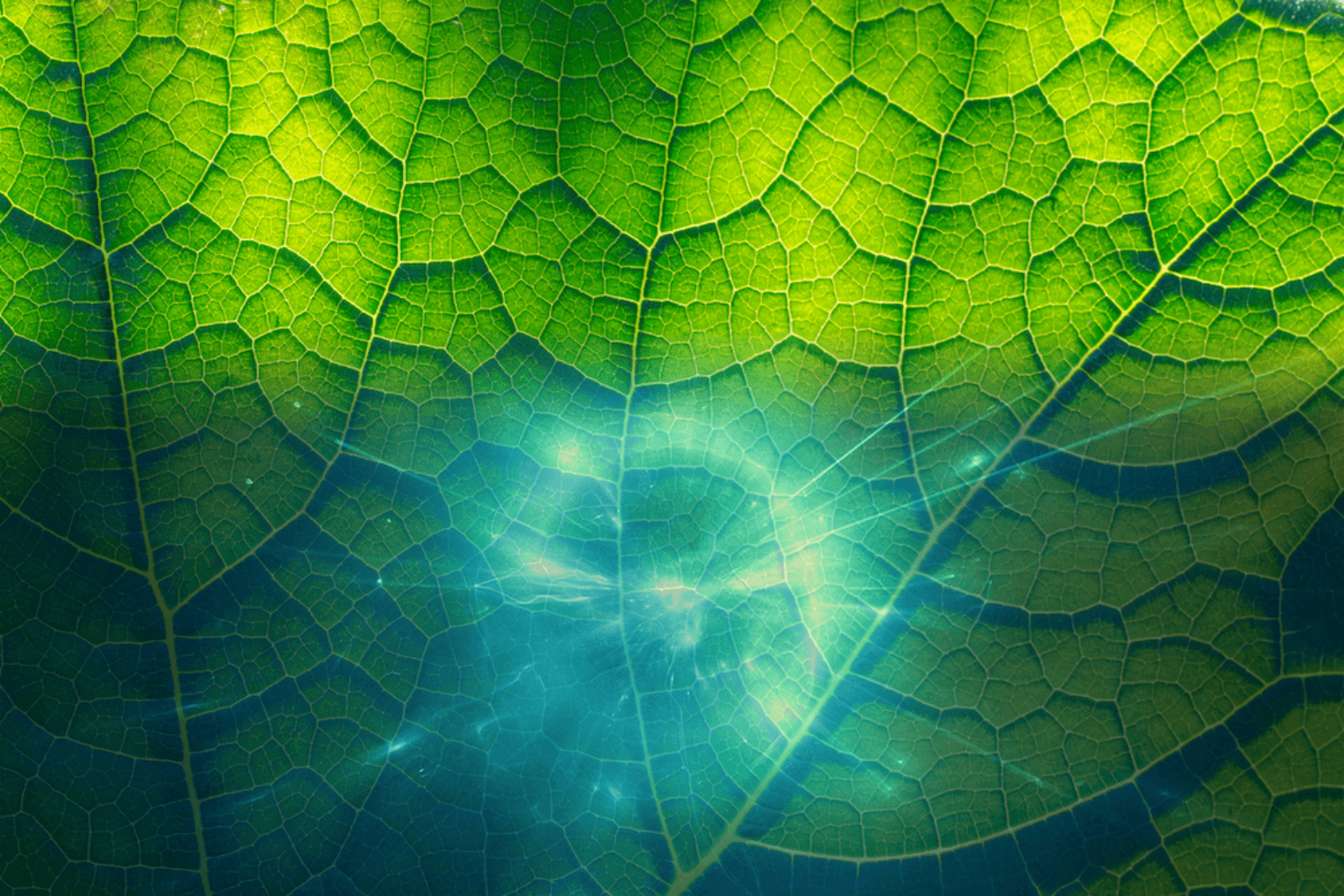
The Author
The words, “quantum mechanics,” evoke visions of laboratories crammed with high-tech gadgetry. Yet quantum mechanics beats in the heart of photosynthesis, the process that makes Earth livable by turning sunlight and carbon dioxide into oxygen and sugars for plant growth.
Although students learn about photosynthesis in school, scientists still struggle to answer fundamental questions. What, for example, makes photosynthesis so highly efficient at turning photons—particles of sunlight—into electrically charged carriers that power photosynthesis’ chemical reactions? How, exactly, do plants absorb photons? Why is the movement of those photons through chlorophyll so much more efficient than through solar cells?
Graham Fleming, a member of the Kavli Energy NanoScience Institute at Berkeley, one of the world’s foremost experts on the quantum mechanical aspects of photosynthesis, has been chipping away at those questions for decades. He believes answering those questions could provide an enormous payoff to society.
“I really want to know how nature works in the early steps of photosynthesis,” he said. “Then we could use that knowledge to create artificial systems that have all of the positive characteristics of the natural system without all the baggage of having to produce seeds, maintain life, or defend themselves against bugs eating them.”
It is a complicated problem, and one he was encouraged as a young researcher not to tackle.
Warm, Wet, and Noisy
After earning a Ph.D. in chemistry from University of London in 1974, Fleming developed high-speed lasers during fellowships at California Institute of Technology, University of Melbourne, and The Royal Institute in London. By the time University of Chicago recruited him to join its faculty, Fleming was using his picosecond (trillionth of a second) lasers to understand what happens during ultrafast chemical processes. At Chicago, when he turned his attention to photosynthesis, his department chair, a very formal theorist, warned him against it.
“He puffed on his pipe and said, ‘These systems are too messy to learn anything from,’” Fleming recalled, meaning they had too many variables to isolate and study in any meaningful way.
“Curiously enough, this didn’t discourage me,” he said. “In fact, it caused me to go off and invent a whole series of new methods to make measurements and progressively learn more and more about these warm, wet, and noisy systems.”
Photosynthesis ranks among the most complex of them and involves myriad molecules and a variety of pigments, each type absorbing a different part of the light spectrum (unlike less efficient solar cells, which absorb mostly ultraviolet rays). Chlorophyll then channels these photons to its reaction center, which taps their energy to transform water and carbon dioxide into oxygen and sugars.
This occurs at very high efficiencies, but no one really understood how. Since the 1930s, physicists had speculated that quantum photons essentially hopped from molecule to molecule like a frog jumping from one lily pad to the next to cross a stream. Others speculated other types of quantum effects might be responsible but could not explain why they lasted as long as they did. Fleming wanted find out.
Waves
His first goal was to learn more the about the composition and structure of the molecules in the photosynthesis system. To do this, scientists typically use spectroscopy, which works by passing white light through a molecule and seeing which wavelengths it absorbs. Each molecule has a unique absorption band signature, which looks like a dilapidated picket fence with most slats missing.
The problem, Fleming explained, is that the signature of the molecules involved in photosynthesis have slightly different spectra. Looking at them together is like looking at picket fences lined up one behind the other. They may all have missing slats, but when viewed head on, they look like a solid wall. “It’s just an impenetrable blob,” Fleming said.
To break through this wall, he invented a technique called ultrafast multidimensional spectroscopy. It pulses femtosecond (one millionth of one billionth of a second) laser blasts at samples, which makes the molecules resonate like a gong struck by a mallet. Each molecule has a distinctive resonance signature, which changes as energetic photons move through it. By observing those changes, Fleming could trace the flow of photons through the system.
What Fleming saw in 2007 astounded him. Instead of photons hopping from one molecule to the next, he saw waves of light coursing through the molecules. This was direct evidence of quantum coherence, which allows light waves to exist in two or more quantum electronic states at the same time. Because quantum mechanics turned each wave into two coherent waves, the light could explore multiple routes to the reaction center, then recombine along the most efficient path.
The waves spread out over several molecules at a time, which explained why they lasted so long. “You play soccer with a big ball instead of a golf ball, because a big ball rolls more easily over a rough field,” Fleming said. “In the same way, if a moving wave spreads out over several molecules, it can average out the imperfections in the system. That makes it more robust and less likely to have some kind of functional failure.”
Yet not everyone was convinced. Some argued that the oscillations lasted too long. Others asked whether the femtosecond laser pulses caused the oscillations and questioned whether they would occur in natural sunlight. To answer those concerns, Fleming needed to dive deeper.
Entangled
Fleming has begun to answer both critiques. Using a new technique called two-dimensional electronic vibrational spectroscopy, he was able to show that photons do more than just move through molecules. They also cause those molecules to vibrate.
“This creates quantum states that are not just purely electronic and not purely vibrational,” he said. “Instead, they’re mixtures.”
This has two effects. First, these hybrid states last longer before the environment breaks them apart. Second, it makes it easier for the photons to jump between fairly large energy differences between molecules.
To study waves in natural sunlight, Fleming teamed with Kavli Energy NanoScience Institute colleague Brigitta Whaley, a highly regarded quantum theoretician with whom he had worked in the past. They soon realized that to answer that question, they had to explain how photons created excited waves in the first place. Did the photosynthesis system store photons until they accumulated enough energy to form a wave or was one photon enough to create an excitation?
This time, Fleming built an instrument that could direct a single photon at a time towards the photosynthesis system. But it was not just any photon. It was a quantum entangled photon, meaning it was one of a pair of photons that behaved as one even when separated. When the researchers aimed one photon at the photosynthesis system, the second photon signaled when it entered.
Knowing this enabled Fleming to make readings at the absolute limit of our ability to measure light. The entangled photons also reveal information about the nature of photosynthesis that is impossible to get without quantum mechanical measurements, he said.
By using a single photon, Fleming can calculate photosynthesis’ true quantum efficiency, rather than estimate its efficiency as averaged over the estimated trillions of photons in even a femtosecond laser pulse. He is also trying to better understand how vibrations improve photon transfer, and how electrons energized by those photons move through molecules.
Dynamic systems
Those electrons boost the energy of oxygen atoms, which rip apart water molecules. Produce too much oxygen, however, and it will begin to destroy the proteins that make photosynthesis possible. To keep this from happening, plants dial back the energy they absorb during the sunniest parts of the day while grabbing every possible photon during dawn, dusk, and on cloudy days.
“The idea that a leaf is just some kind of green solar panel is very far from the truth,” Fleming said. “It’s highly regulated and dynamic, and it reacts on timescales of passing clouds or even the flickering of other leaves on a tree in the wind.”
Fleming hopes he can learn enough about the principles underlying photosynthesis to make solar cells more efficient. He is also teaming up with UC Berkeley colleague Krishna Niyogi, a plant biologist, to do the same with plants.
Plant regulation systems react to bright sunlight quickly, but take 10 to 15 minutes to resume full production when the sun goes behind a cloud. If they could spring back faster, plants would become more efficient and grow faster. His goal is to understand how the regulatory system works so Niyogi can genetically reengineer plants to achieve higher efficiency levels.
It is an ambitious goal. But so was exploring the chemistry and physics of warm, wet, and noisy systems. “It is enough,” Fleming said, “to keep anyone busy for a long time.”