Living the Good Life on Mars
by Alan S. Brown
A nanofiber-bacterial system, a tiny fuel cell powered by sweat, cheaper sensors, and artificial skin
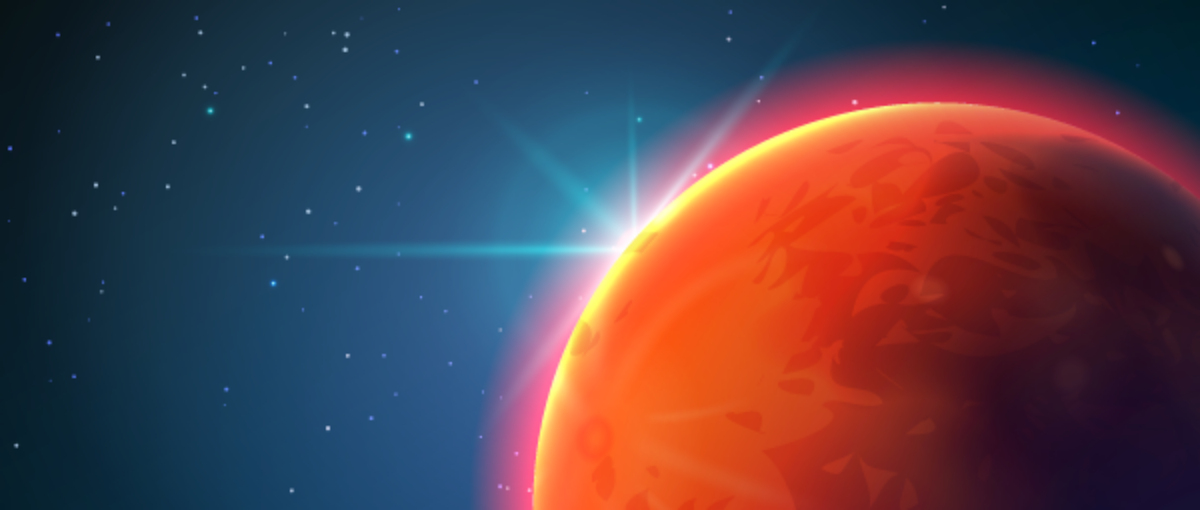
The Author
The Researchers
The mechanisms of life take place at the nanoscale. Studying them allows us to understand how and why living things behave the way they do. It also enables us to harness those mechanisms to interact with nanoscale machines and sensors. Here we will look at everything from a hybrid nanofiber-bacterial system to make drugs and plastics on Mars to a tiny fuel cell that uses only the chemicals found in sweat and oxygen from the air for power. We will also look at tiny, cheap sensors that can be produced by the millions and why stretchiness is important for artificial skin.
Living the good life on Mars

When humans get to Mars, they will need to make everything from pharmaceuticals and disinfectants to plastic chairs and fuels. Peidong Yang, director of the Energy Nanoscience Institute at Berkeley, has been working on this problem for eight years. His unusual solution: a combination of silicon nanowires and bacteria that makes acetate from carbon dioxide and water. Mars is the perfect place for this reaction, since 90 percent of the atmosphere is 90 percent carbon dioxide and water appears abundant. Acetate, a two-carbon molecule based on vinegar, is easy to upgrade chemically into a wide range of useful products. In his most recent work, Yang boosted efficiency—the amount of solar energy converted into carbon bonds—to 3.6 percent. That is nine time better than the system Yang demonstrated five years ago, better than most plants, and close to cane sugar, the most efficient of all plants at 4 to 5 percent.
Skin sensor does not break a sweat
Many researchers want to apply flexible sensors to the body to monitor heart rate, temperature, blood sugar, metabolic byproducts that indicate health, and even the nerve signals that control our muscles. Nearly all these inventions have one flaw: power. They need power to drive their sensors and the Bluetooth transmitter they need to send the data they collect to a smartphone app. This usually involves pairing a nearly invisible flexible sensor with a bulky battery (or solar cell or motion energy-harvesting device). Wei Gao, an affiliate of the Kavli Nanoscience Institute at Caltech, has a more elegant solution. He has developed an “e-skin” fuel cell that turns sweat into power. It works by combining lactate, a byproduct of digestion and exercise that is found in sweat, and oxygen from the air into water and pyruvate, another metabolic byproduct. This reaction generates enough power to power the sensors and a Bluetooth transmitter.
Paramecium-sized sensors by the millions

Venting in the deep blue sea
Can a partnership between two types of bacteria—or bacteria and worms—on the deep ocean floor stabilize Earth’s atmosphere and climate? Victoria Orphan, an affiliate of the Kavli Nanoscience Institute at Caltech, a 2016 MacArthur Fellow, and a recent inductee of the American Academy of Arts and Sciences, believes so. Her work focuses on how bacteria and archaea work together to consume up to 80 percent of the methane released from vents on the ocean floor. This keeps the methane from bubbling up into the atmosphere and heating the earth. Her latest work explains why deep-sea worms, which do not live on methane, congregate near underwater methane vents. It turns out that methane-eating bacteria live on the worms’ lungs. The worms give them a perfect environment to grow and the bacteria in turn provide a diet for the worms.
What makes collagen stretch?
The protein collagen acts like glue to hold together our skin, bones, muscles, cartilage, ligaments, and hair. Yet its elasticity varies greatly, from the stretchiness of skin to the relative solidity of the cartilage in our nose. Gijsje Koenderink, a member of the Kavli Institute of Nanoscience Delft, has been working to understand what makes collagen strong. Her research shows that collagen fibers can organize themselves in different ways, from strong rope-like fibers in tendons to disorderly but highly flexible networks in skin. The more connected those networks, the stiffer the collagen. Mastering those connections could one day help researchers grow better replacement skin for burn victims, or perhaps create networked materials that change color as pressure rises or that can heal themselves.
